Thermodynamic and Structural Cu-Sn-Zn Alloy Properties
Thermodynamic and Structural Cu-Sn-Zn Alloy Properties
Abstract
Direct experimental observation of partial (for each of the three components) and integral thermodynamic functions in the Cu-Sn-Zn system at near-room temperature range has been performed. A significant difference between the properties of the "equilibrium" and hardened specimens has been found. This fact has been validated by the difference in their structural conditions and phase states. Determination of thermodynamical properties is required for developing copper-bearing alloy recycling technologies. The alloy under investigation features a negative formation of Gibbs energy. It shows a relative thermodynamic stability of its state at the experimental temperatures. The hardened specimens feature an excess Gibbs energy compared to "equilibrium" annealed alloys of the same chemical composition. It enables the evaluation of the "non-chemical" macrostructure contribution into the thermodynamic properties of the Cu-Sn-Zn alloys.
1. Introduction
New developments in engineering and manufacturing technology require a wider choice of structural materials and alloys containing non-ferrous, noble and rare metals. The downsides of such a development are ore deposits depletion and price hikes, raising energy consumption in mining industry, and a constantly expanding share of hard-to-recycle waste. In this situation, recycling metal scrap and other man-made waste in order to extract valuable non-ferrous components has become relevant. There are promising hydrometallurgical and electrochemical processes for making copper-bearing alloys from recycled resources. Such processes extract several components at once, while the capital investments are low [1]. One of the primary tasks in developing the theoretical foundation of the processes is performing thermodynamic and kinetics studies to find out the behavior of copper-bearing multicomponent alloys as recyclable materials. To develop a manufacturing process it is important to determine the relation between the temperature, composition, microstructure, electrolyte properties and hydrometallurgical and electrochemical process parameters [2]. Phase changes occur in such materials under heat treatment; for instance, these martensitic transformations leading to a non-equilibrium state of the system. A thermodynamic study of the alloys is of practical importance in order to identify the optimal operation modes and tendency to relaxation. In general, it should be emphasized that a targeted approach to tailored material development requires the availability of its thermodynamic properties and their relations vs. the substance structure. At the same time, thermodynamic properties are fundamental for research since they express a quantitative measure of atomic interactions between components in alloys and compounds, determine the phase equilibrium nature and its evolution as external conditions change [3].
The target of research is a triple Cu 87.1%, Sn 8.8%, Zn 4.1% (at. %) system alloy. The alloy features a β → β'' martensitic transformation [4]; it is widely used as a multicomponent anode for electrolytic refining. The paper [5] proves the existence of a direct relation between the chemical potentials of the alloy components and the corresponding electrode potentials, both in homogeneous and heterogeneous Сu-based metallic systems. It has been found [5] that under selective anodic dissolution the activity of copper at the phase interface exceeds one (aCu>1), i.e. it is higher than the pure metal's activity. The surface layer is a metastable system, capable of further spontaneous transformation into a thermodynamically stable phase. We have observed higher thermodynamic activity of the components in non-equilibrium (hardened) Fe-Ni, Cu-Ni-Al, Fe-Co-Si-B and some other specimens [3].
Till now, there have been virtually no direct experimental data available on the thermodynamic properties (such as component activities) of Cu-Sn-Zn alloys at near-room temperatures. For that reason, the research object is the determination of partial and integral thermodynamic functions in the Cu-Sn-Zn system vs. temperature, and finding the relations between heat treatment modes and thermodynamic and structural properties.
2. Phase and Structural State
The Сu-Sn-Zn alloy phase equilibrium diagram has been studied for Cu-rich alloys [6].
At 500о С(Fig. 1)the γ (Сu-Sn) and β (Сu-Zn) phases are in equilibrium with the solid FCC Cu-based (α phase) solution. The dashed line is the α / α+ γ boundary position at 300о С.
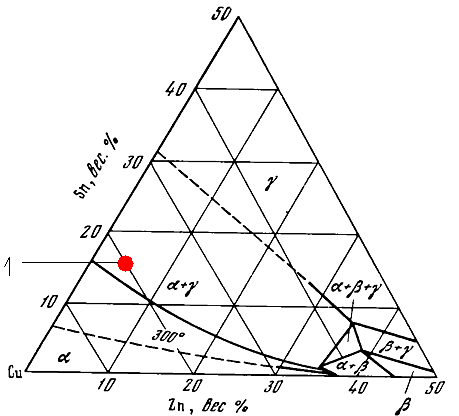
Figure 1 - Isothermic cross-section of the Cu-Sn-Zn equilibrium diagram at 500о С
Point 1 as marked on the figure corresponds to the composition of the Cu-Sn-Zn alloy under investigation (Cu: 80.0 mas. %, Sn: 15.6 mas. %, Zn: 4.4 mas. %). Below 300о С the γ phase transforms into the ε phase or δ phase. The temperature of the δ phase decomposition into the α + ε eutectoid is about 380оС in the Cu-Sn binary alloy [7]. However, this transformation is very slow. In most cases, the δ phase is stable below 350 оС.
We have studied some Cu-Sn-Zn alloy specimens having the same chemical composition but exposed to different heat treatment modes. The alloy has been manufactured at Bardin Ferrous Metals Research Institute by air melting with cryolite slag; electrolytic copper, chemically pure stannous and zinc have been used. The intersitial impurities content like carbon, sulphur, and phosphorus has not exceeded 0.01 mas.% and 0.04 mas.% for ferrous. The impurity composition and content have been measured at the Metallurgy Dept., Tula Arms Factory, with X-ray fluorescence spectroscopy using a S1 TURBOsdLE analyzer. We performed the subsequent heat treatment and the phase composition, structure and thermodynamic properties study.
To make a material at a near-equillibrium state, the initial alloys have been annealed in a vacuum quartz tube at 300оС with subsequent slow cooling in the furnace. Another heat treatment used has been a hardening by heating up to 700оС and cooling with water-ice mixture and subsequent extra cooling with liquid nitrogen. Such a treatment could have produced a β phase being equilibrium at the given temperature (700оС) with its subsequent hardening and possible martensitic transformation.
The X-ray phase analysis of the Cu-Sn-Zn specimens has been performed with a DRON 2 diffractometer using CoKα radiation; the quantitative metallographic analysis has been performed with a Neophot 2 microscope. The specimens have been etched with a custom-composition etch.
The analyses have shown that the annealed specimen has a two-phase α + ε structure with the α and ε phases FCC lattice and contains 8 vol. % of the ε phase. The hardened alloy is also a two-phase one, consisting of the α and β'' phases (8 vol. % of the β'' phase; β'' is a martensitic metastable phase with orthorombic structure.
3. A Thermodynamic Study
Thermodynamic Properties of Cu-Sn-Zn Alloys
The thermodynamic properties of Cu-Sn-Zn alloys have been determined using the previously developed methods applicable to non-equilibrium specimens [3], [8]. The primary method is a version of the EMF method: the Touch Instant EMF method (TIE) [9], [12].
To register the EMF we have used a GDS-806C general-purpose digital oscilloscope.
The TIE method has been applied to the experimental thermodynamic research of the Cu-Sn-Zn alloy with the following concentration element types:
(-) Cu, Cu │CuSO4, aq│Cu-Sn-Zn, Cu(+) (1)
(-) Cu, Sn│SnCl2, aq │Cu-Sn-Zn, Cu (+) (2)
(-) Cu, Zn│ZnSO4, aq│Cu-Sn-Zn, Cu (+) (3)
(-) Cu, Cu-Sn-Zn (hardened)│CuSO4, aq│Cu-Sn-Zn (annealed) Cu (+) (4)
(-) Cu, Cu-Sn-Zn (hardened)│ SnCl2, aq │Cu-Sn-Zn (annealed) Cu(+) (5)
(-) Cu, Cu-Sn-Zn (hardened)│ZnSO4, aq│Cu-Sn-Zn (annealed) Cu (+) (6)
Water or water-glycerin solutions of the corresponding salts have been used as electrolytes. The electrolyte concentration range has been 0.1М…1М. The measurement method is covered in detail in the monograph [3].
The EMF values for the elements (1-3) vary directly with the difference of the components' chemical potentials in the alloyed electrodes (Dmi = - ziFE), where z is the ion charge of the potential-determining component (in this case for all the elements z =2); Δμi = μi - μio and corresponds to the difference of the components' chemical potentials in the alloy and a pure metal (i.e. in the standard state). F is the Faraday constant, E is the mean EMF value.
Table 1 - Relation between Chemical Potential Variations in the Cu-Sn-Zn Alloy and the Temperature
Т, К | 295 | 313 | 333 |
ΔμCu, kJ/g-at. | - 0,53 ± 0,07 | - 0,74 ± 0,08 | - 0,82 ± 0,06 |
ΔμSn, kJ/g-at. | - 6,20 ± 0,25 | - 7,12 ± 0,30 | - 7,87 ± 0,30 |
ΔμZn, kJ/g-at. | - 10,90 ± 0,29 | - 13,47 ± 0,40 | - 14,26 ± 0,35 |
Table 2 - Calculated Partial Enthalpy and Entropy of Formation for the Cu-Sn-Zn alloy
|
|
|
Cu | 8 ± 2 | - 3,2 ± 1,0 |
Sn | 36 ± 4 | - 15,1 ± 3,5 |
Zn | 58 ± 8 | - 28,5 ± 4,5 |
All the integrated thermodynamic functions for the Cu-Sn-Zn alloy have been calculated as follows:
For instance, the Gibbs energy of formation the triple Cu-Sn-Zn alloy from its components has been determined as:
ΔfG = xCu ΔμCu + xSnΔμSn + xZn ΔμZn
Table 3 - Gibbs energy of Formation the triple Cu-Sn-Zn alloy vs. temperature
Т,К | 295 | 313 | 333 |
Δ f G, kJ/mole | - 1,46 ± 0,15 | - 1,82 ± 0,20 | - 1,99 ± 0,20 |
The integral enthalpy and entropy values for the Cu-Sn-Zn alloy have been evaluated as follows:
ΔfS = 13 ± 3 J/g-atom∙К and ΔfH = - 5.3 ± 1.5 kJ/g-atom
The excess Gibbs energy value, i.e. its variation as a solution is formed from the pure components over the ideal solution value has been calculated as follows:
The result is = - 0.5… - 0.8 kJ/mole, showing a small negative deviation from the ideal solutions law. Such a deviation indicates a relative stability of the specimens' structure while the small formation entropy value indicated a possible inclusion of a new ε phase, compounds (i.e. Cu5Sn, Cu4Sn) and other intermetallides in the α solid solution [5], [6], [7].
Determining the Difference of Thermodynamic Properties in the Hardened and Annealed Specimens
All the above given thermodynamic data are for the annealed (equilibrium) Cu-Sn-Zn alloy specimens. Using the galvanic cell EMF values (4-) we have managed to directly calculate the difference of the components' chemical potentials and the difference of the Gibbs energies in the hardened and annealed Cu-Sn-Zn alloy specimens of the same chemical composition at Т = 295 К (see Table 4) with the following equations:
and
Table 4 - Difference of the Components' Chemical Potentials and the Gibbs energies in the hardened and annealed Cu-Sn-Zn alloy specimens at Т = 295 К (J/mole)
|
| |
|
480 ± 100 | 2390 ± 150 | 1850 ± 140 | 710 ± 90 |
The calculated ≈ 700 J/mole value corresponds to the Gibbs energy difference in the hardened specimens, containing 8 vol. % of the martensitic β' phase, and the annealed alloys of the same chemical composition that contain the ε phase. In both cases, the primary phase component is the solid α solution. The slightly increased Gibbs energy value in the hardened specimens is probably due to the positive "non-chemical" contribution into their Gibbs energy from the internal stress and various defects caused by both fast cooling and the martensitic transformation. The hardened specimens under the thermodynamic experiments have been in a non-equilibrium state, so some extra (internal) parameters are required to describe it. For example in the Fe-Ni and Mn-Cu systems we have used (ξ), the martensitic phase part of the austenite-martensite system [3], as one of such parameters. So, the obtained
≈ 700 J/mole value corresponds to ξ = 0.08. By extrapolating the ΔG = f(ξ ) relation [3] to ξ = 1.0 (100 % of the martensitic phase) we obtain
≈ 4000 J/mole. It is close to similar values in systems with non-thermoelastic martensitic transformation, like Fe-Ni.
4. Conclusion
The direct galvanic cell EMF measurements have enabled the determination of their equilibrium values and calculate the partial thermodynamic functions for the Cu-Sn-Zn alloys. Since the partial values have been determined for all three components, the calculation of the integrated thermodynamic properties has also been carried out. The measurements have been taken at several temperatures to determine the partial and integral formation enthalpy and entropy.
The alloy under investigation features a negative formation Gibbs energy. It shows a relative thermodynamic stability of its state at the experimental temperatures.
The hardened specimens feature an excess Gibbs energy compared to "equilibrium" annealed alloys of the same chemical composition. It enables the evaluation of the "non-chemical" macrostructure contribution into the thermodynamic properties of the Cu-Sn-Zn alloys.
The Touch Instant EMF method also facilitates the thermodynamic properties study in hardened metallic alloys at low temperatures, while preserving their non-equilibrium state required for many engineering applications.