Application of Nanosecond Microwave Pulses in Batch Regime for Healing of Burn Wounds
Application of Nanosecond Microwave Pulses in Batch Regime for Healing of Burn Wounds
Abstract
This study focused on the development and assessment of a small, low-intensity generator of nanosecond microwave pulses for life science applications. A microwave source based on a magnetron with an operating frequency of 9.4 GHz makes it possible to obtain stable generation of pulses with a duration of 100–500 ns. The device has been tested in biological experiments investigating the effect of RPMs on thermal wound healing. It has been shown that fourfold exposure to nanosecond microwave pulses under certain parameters (peak power flux density of 140 W/cm2, pulse repetition rate of 8 Hz) provides effective wound healing in laboratory rats. With the help of computer simulation, a picture of the propagation of microwaves was obtained, the characteristics of the incident wave were found, and estimates of the specific absorption rate were given. Obtained results can be taken into account when developing devices for therapeutic and cosmetic cabinets.
1. Introduction
New sources of pulsed radiation, such as generators of nanosecond repetitive pulsed microwaves (RPM) based on accelerated electrons, have come in use in various fields of science. They feature short pulse durations (tens of nanoseconds) and high pulse powers. This type of radiation in packet regime has been shown to be capable of producing high biological effects in various types of cells and tissues , . It was established that nanosecond microwave pulses demonstrated high efficiency in inhibiting tumor cells, stimulating stem cell proliferation , , stimulating ulceration of the gastric mucosa healing , as well as stimulating skin wound healing . Apparently, RPM has a high promise for healing wounds of a certain etiology.
One of the important tasks of practical medicine is to accelerate the healing of burn wounds. This is relevant because, according to WHO; every year around the world, people receive 11 million different burn injuries, 300,000 of which are fatal . Due to developing cicatricial contractures and complications, up to 2.3% of patients become disabled, and about 40% of patients with deep burns need further reconstructive and restorative operations. Current approaches to the treatment of burn injuries (using surgical procedures and options for antimicrobial therapy and care) are not effective enough; therefore, additional attempts are being made to use other practices, in particular physical factors. These factors include laser radiation , , , , magnetotherapy , , photodynamic therapy , as well as pulsed electromagnetic radiation , . A special place among the physical factors is occupied by pulsed sources of radiation. To date, a large amount of data has been accumulated on the higher biological efficiency of precisely pulsed organization of irradiation compared to continuous field generation with equal energy characteristics , , . The high biological efficiency of low-intensity electromagnetic action in the case of generating pulses with nanosecond duration is not associated with thermal action; in this case, the information organization of the action is of great importance. Living organisms respond to information encoded in the physical (modulation) or temporal parameters of exposure (radiation intensity, pulse delivery rhythm). According to W. Adey, there are frequency "windows" of low-intensity electromagnetic exposure, within which the biological effect is most pronounced: the modulation frequency range is from 6 to 20 Hz . In the works of some researchers, this frequency range also turned out to be biologically effective , , , .
Although various physical factors are capable of accelerating wound healing, they nevertheless have a number of disadvantages. In particular, they are technically complicated and cumbersome, the exposure procedure can take a long time and be accompanied by a significant energy load on the body, and to one degree or another, have an undesirable effect. Therefore, the most promising should be factors that have high biological efficiency with minimal energy load on the body and do not have negative consequences. These requirements are best met by nanosecond RPM. In accordance with this, the source of nanosecond microwave pulses must be able to vary its characteristics (amplitude, pulse repetition rate, number of pulses per session, number of sessions) in order to provide the necessary positive effect for a particular patient within the framework of the requirements of personalized medicine. Such an RPM source should have a low output power to provide low-intensity exposure, be compact and highly mobile for convenient use in physiotherapy and cosmetology clinics, and provide comfortable conditions for patients. All these requirements can be met by using a low-intensity, small-sized source of nanosecond microwave pulses.
The low average power of microwave radiation is due to the maximum SAR (Specific Absorption Rate). This is directly related to safety for the irradiated object and personnel. Increasing the absorbed power increases the specific absorption rate. SAR is determined :
where PL is the power of losses in the medium, ρ (kg/m3) is the density of the substance, and E(V/m) is the electric field strength, σ is the conductivity of the medium.
The paper presents the intermediate results of studies conducted with laboratory rats. The objects under study were irradiated with microwave pulses with a carrier frequency of 9.4 GHz and a duration of about 100 ns. During the next four days after the application of the burn, laboratory rats were subjected to a single exposure to nanosecond microwave pulses (up to 4000 pulses per session/day) with an intensity of 140 W/cm2 at a pulse repetition rate of 8 Hz. As a result, a positive effect was obtained and modes with a minimum radiation dose were found, which lead to accelerated healing of burn wounds.
To generate microwave radiation, a compact device based on a magnetron was developed with the ability to adjust the output power, pulse duration, and pulse repetition rate. It also includes a waveguide system and a horn antenna. The description and characteristics of the generator are presented in this article. As an object for testing the biological effectiveness of the small-sized generator of nanosecond microwave pulses considered in the article, an experimental model of a third-degree burn on the dorsal surface of ‘Wistar’ laboratory rats was used. The traditional way for a burn model is to use a metal rod , . There were used biological characteristics for estimation: the area of the burn (wound area), the number of formed elements of the peripheral blood of rats, histological analysis of skin samples 30 days after the formation of the burn wound.
2. Research methods and principles
2.1. Experimental objects. Generator
A complex based on the MI-459 magnetron has been developed for medical and biological research. Magnetron MI-459 pulse action, with a non-incandescent cathode, auto electronic triggering, packaged, magnetically shielded design, 3 cm wavelength range, operating at a fixed frequency, output pulse power of at least 20 kW with a pulse duration of 0.05-1.0 μs, designed to work as a generator of high-frequency energy in the equipment for national economic purposes.
Fig. 1 shows the scheme for fulfilment research using a microwave generator. Generation frequency 9.4 GHz. Pulse repetition frequency from 1 Hz to 2000 Hz. The duration of pulses at half power level is about 100 ns. It is possible to adjust the power over the entire range using a tunable attenuator. A standard 23 mm x 10 mm rectangular waveguide is used to transport microwave radiation. At the output of the waveguide path, there is a horn pyramidal antenna with a horn opening of up to 90x40 mm.
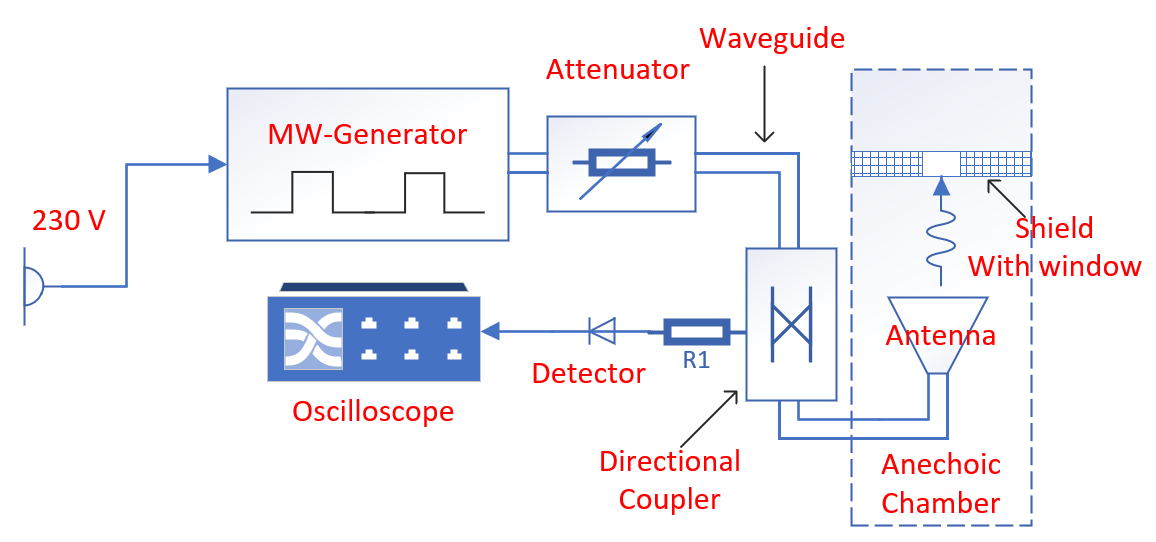
Figure 1 - Scheme of an experimental stand for biomedical research
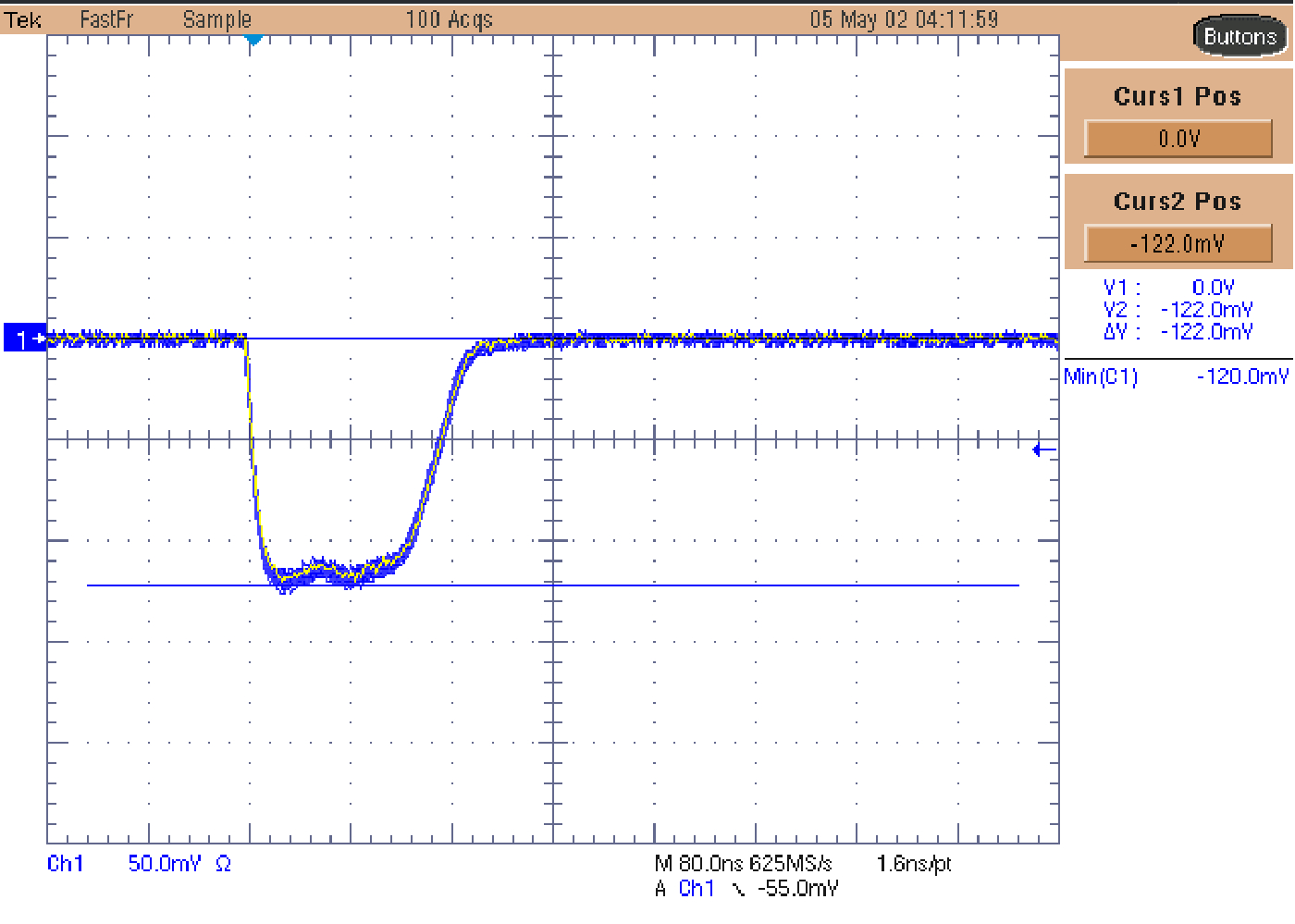
Figure 2 - Oscillogram of detected signals in the Fast Frame mode of 50 pulses
Note: vertical scale 50 mV/div, horizontal scale 80 ns/div
The working space in which irradiation is carried out is an anechoic chamber in which the walls are covered with radio-absorbing material in order to minimize the influence of standing waves.
The reflectance of this material was determined in another experiment using a standard method using an antenna and an Agilent PNA N5227A panoramic network analyzer. At an operating frequency of 9.4 GHz, its value is -27 dB.
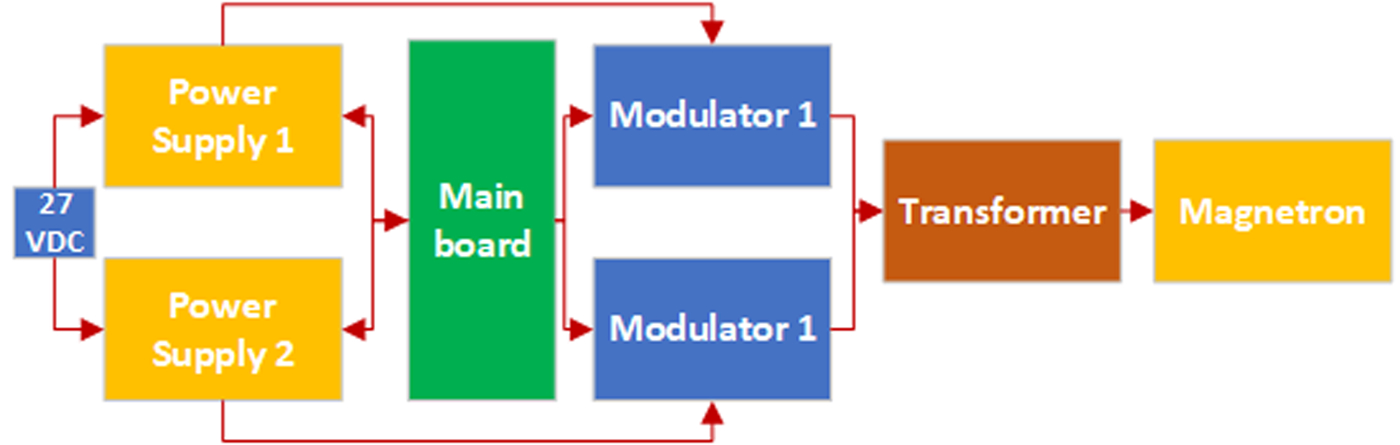
Figure 3 - Block diagram of the MW-generator
where Pref is the power reflected from the boundary of air and biological tissue (part of which falls back into the horn, the rest is scattered on the chamber walls); PTloss is the power absorbed in the biological tissue; PCWloss are the remaining power losses associated with the wave incident on the chamber walls, including those due to the existence of side lobes in the diagram.
Estimating the incident, reflected, and absorbed power under these experimental conditions is a non-trivial task. This is due to the difficulties of measuring the power flow in the near field of the radiating antenna. A typical technique for determining the power flux-density distribution using a receiving antenna gives a significant error due to the fact that the phase front of the wave has a spherical surface. In this case, the receiving antenna begins to influence the radiation pattern by changing the main lobe and increasing the side lobes in it. It is for this reason that, in our opinion, 3D computer numerical modeling becomes the most suitable tool for estimating these parameters.
The irradiated animal was fixed behind a screen, which consisted of an absorber about 25 mm thick. To output microwave radiation to a biological object, the screen has a rectangular output window 70 mm x 45 mm. A screen with a window allows irradiation of only a certain area of the animal without exposing the rest of the body to microwave exposure.
The distance from the antenna opening to the surface of the animal was set equal to 200mm.
The experimental study has been performed on 20 mature female Wistar rats with mass of 230-250 g. The animals were kept in standard vivarium conditions under natural light regime and on a standard diet with free access to water and food. All procedures with animals were performed at the same time (from 9:00 to 11:00). The study was performed in accordance with the ethical standards of work with laboratory animals and the sanitary rules for the design, equipment and maintenance of experimental biological clinics . The research has been performed on the basis of permission of the Bioethics Commission of the Biological Institute of the National Research Tomsk State University, Tomsk, Russia (Protocol No. 46 from 29.05.2023).
Experimental animals have been randomly divided into two groups of 10 individuals:
1 The control group (n=10) – the rats which after modeling the thermal burn were kept in standard vivarium conditions and not exposed to RPMs. In these rats, burn injuries healed on their own;
2. The experimental group (n = 10) – the rats which after modeling the thermal burn were subjected once in 4 days to local exposure of nanosecond RPMs with pPFD (peak power flux density) of 140 W/cm2 and pulse repetition rate of 8 Hz (mode in which previously RPMs showed the greatest regenerative efficiency) , .
2.2. Modeling and irradiation of thermal burns. Modeling of thermal burns
One day before modeling, burn on the dorsal surface of rat's body the skin area was depilated by shaving with an electric veterinary machine. Shaving and burn modeling were performed under CO2 anesthesia. The thermal wound was created by applying a metal rod (Fig. 4) with diameter of 2 cm heated up to 100°C to the skin surface in the interscapular region without effort for 30 seconds .
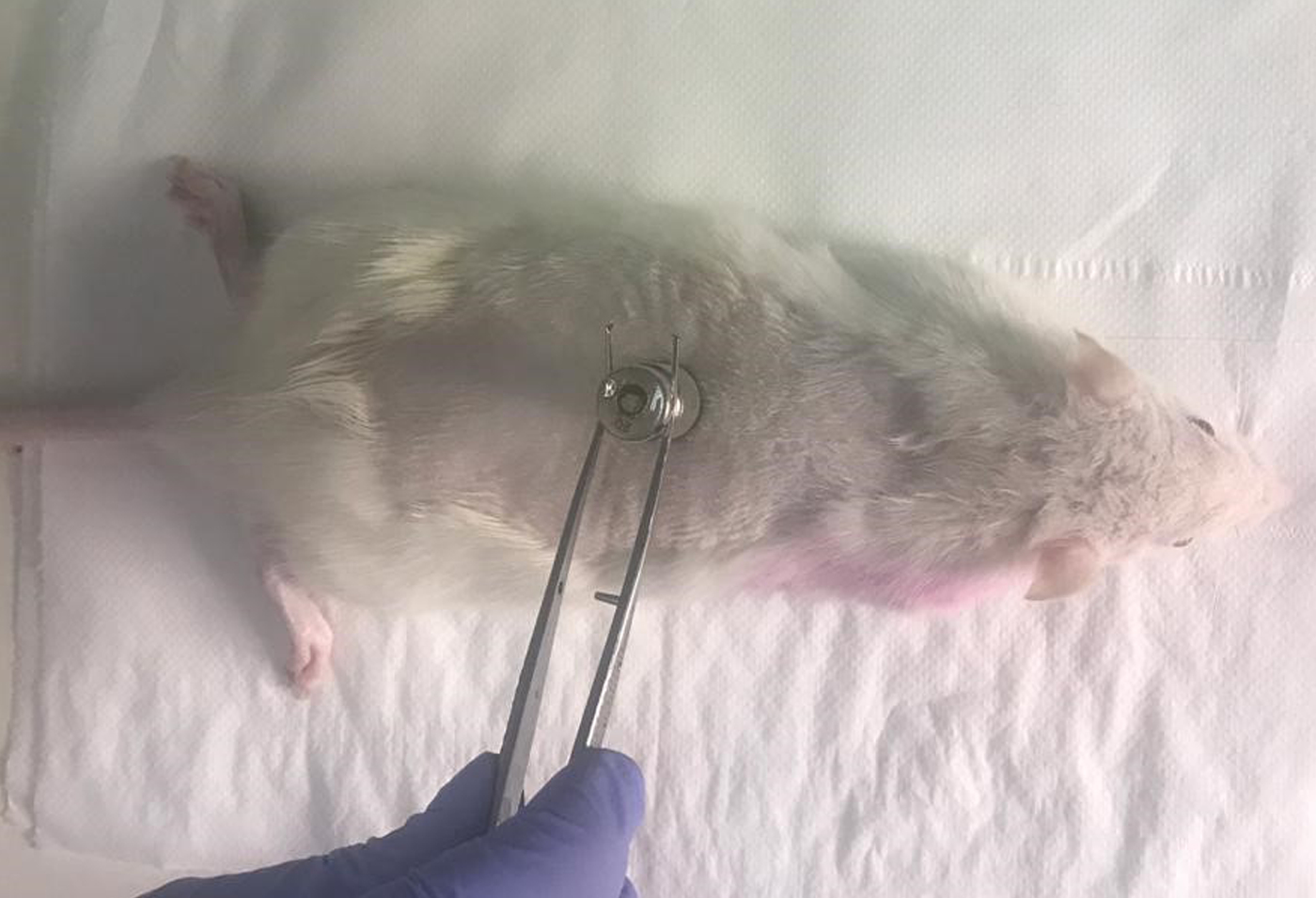
Figure 4 - Modeling of thermal injury in rats
The wound healing process was assessed by reducing the surface area of the wound defect in dynamics on days 1, 5, 14, 19, 24 and 28 after the burn simulation . Visual fixation of the affected area was provided using a Sony-DSC-F717 camera (Japan). Further, the obtained images were analyzed using the “Imageanalyzer” software package. The obtained values of the area of burn wounds in irradiated animals were compared with similar indicators in the control group. In all the studied groups, the timing of the fall of the primary scab was recorded, the presence / absence of inflammation in the wound area was visually recorded.
2.3. Irradiation of thermal burns
In 5 hours after formation of burn the laboratory rats were exposed daily for 4 subsequent days to single exposure of nanosecond RPMs (4000 pulses per session/ day) with intensity of 140 W/cm2 at pulse repetition rate of 8 Hz (Fig. 5). The used peak intensity was recorded according to the standard method based on antenna measurements and calorimetric calibrations . At the used pulse repetition rate of 8 Hz and radiation intensity of 140 W/cm2, the heating at the burn surface after exposure to 4000 pulses did not exceed 0.2°C. Based on the obtained temperature growth rate, the value of the specific absorbed power (SAR) was calculated, which was about 1.0 W/kg.
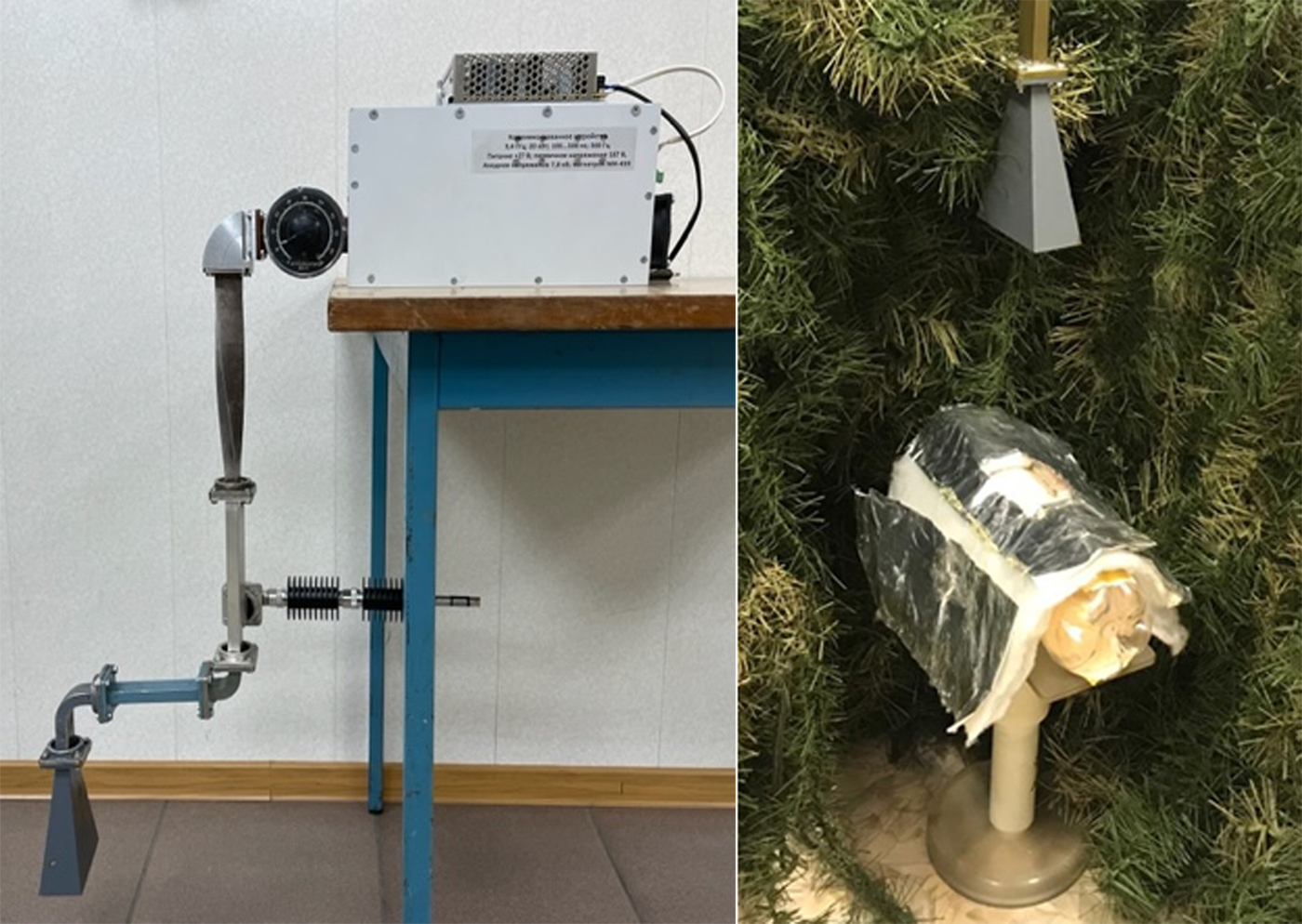
Figure 5 - Stand for location and irradiation of a biological object
2.4. Analysis of the exposure
2.4.1. Analysis of hematological parameters
During the study, the peripheral blood of rats was analyzed every 5–7 days (hemoglobin content, the number of erythrocytes, platelets, leukocytes, and their subpopulations) using a PCE-90 Vet veterinary hematological analyzer (High Technology, USA). Blood sampling was carried out under CO2 anesthesia by incising the gums into a Microvette tube (tri-Kallium-EDTA, Germany).
2.4.2. Histological analysis
On the 30th day after the simulation of thermal burns, the animals were taken out of the experiment by an overdose of CO2 anesthesia. For histological analysis, skin sections were obtained, including both the affected and adjacent (intact) areas. Histological processing of the material was carried out according to standard methods , , . Slices microscopy, imaging of selected representative samples, and measurements were carried out using an AxioLab A1 microscope, an AxioCamERc 5s camera, and the ZEN 2 program (Carl ZeissMicroscop, Germany).
Micrographs were used to measure the total area of the wound (AF, µm2), the area of granulation tissue (AGT, µm2), the area of ruptures in the studied fragment (AR, µm2), the thickness of the newly formed epidermis (TE, µm) or the wound length – the distance between the edges of the wound (WL, µm) – for wounds with incomplete epithelialization. Based on the primary measurements, the relative area of granulation tissue (RAGT) was calculated:
TE, LW, RAGT indicators were used to compare the experimental groups.
2.4.3. Statistical analysis of data
Statistical processing of the obtained results was carried out using the capabilities of the program Statistica 8.0 for Windows. The results obtained were presented as the arithmetic mean and the standard error of the arithmetic mean (M ± m) for all groups of experimental animals over time. The significance of differences in values between the control and irradiated parameters was determined using the nonparametric Mann–Whitney U test. The critical level of significance of differences p in testing statistical hypotheses was taken equal to 0.05. For statistical processing of the results of histological analysis, the following were calculated: the mean value (x̅), minimum (min) and maximum (max), standard deviation (σ), standard error of the mean (mx̅), coefficient of variation (Cv), significance level (p). To assess the nature of the distribution, the Kruskal–Wallis test was used. To compare the results of the groups, Fisher's exact test and Student's test were used. When testing statistical hypotheses, a significance level of less than 5% (p < 0.05) was considered significant.
3. Main results
In the study, all experimental rats had a IIIA (by ) degree burn, characterized by necrosis of the epithelium to the depth of the epithelial layer with the preservation of skin appendages.
In rats of the control group, the healing of thermal wounds was a consistent monotonous decrease in the area of wounds from days 1 to 30 of the study (Fig. 6). At the same time, a long-term preservation of the scab (up to 16 days of the experiment) was observed in control animals. According to the results of histological analysis of the skin of rats in the control group, complete epithelialization of wounds was recorded in 33% of the animals after 30 days (Fig. 6, 7).
In rats of the experimental group after 4-fold local exposure to nanosecond microwave pulses with an intensity of 140 W/cm2, the initial stages of the dynamics of burn healing did not differ from the control group. However, by day 5 after the burn simulation, the effect of reducing the area of the wound in the experimental group was 15% (Fig. 6). From the 19th day of the experiment, the healing acceleration effect was 76%, and by the 24th day, the healing acceleration effect was 96% relative to the control group. According to the results of histological analysis, by the 30th day of the study, 67 % of the animals of the experimental group had complete epithelialization (Fig. 7).
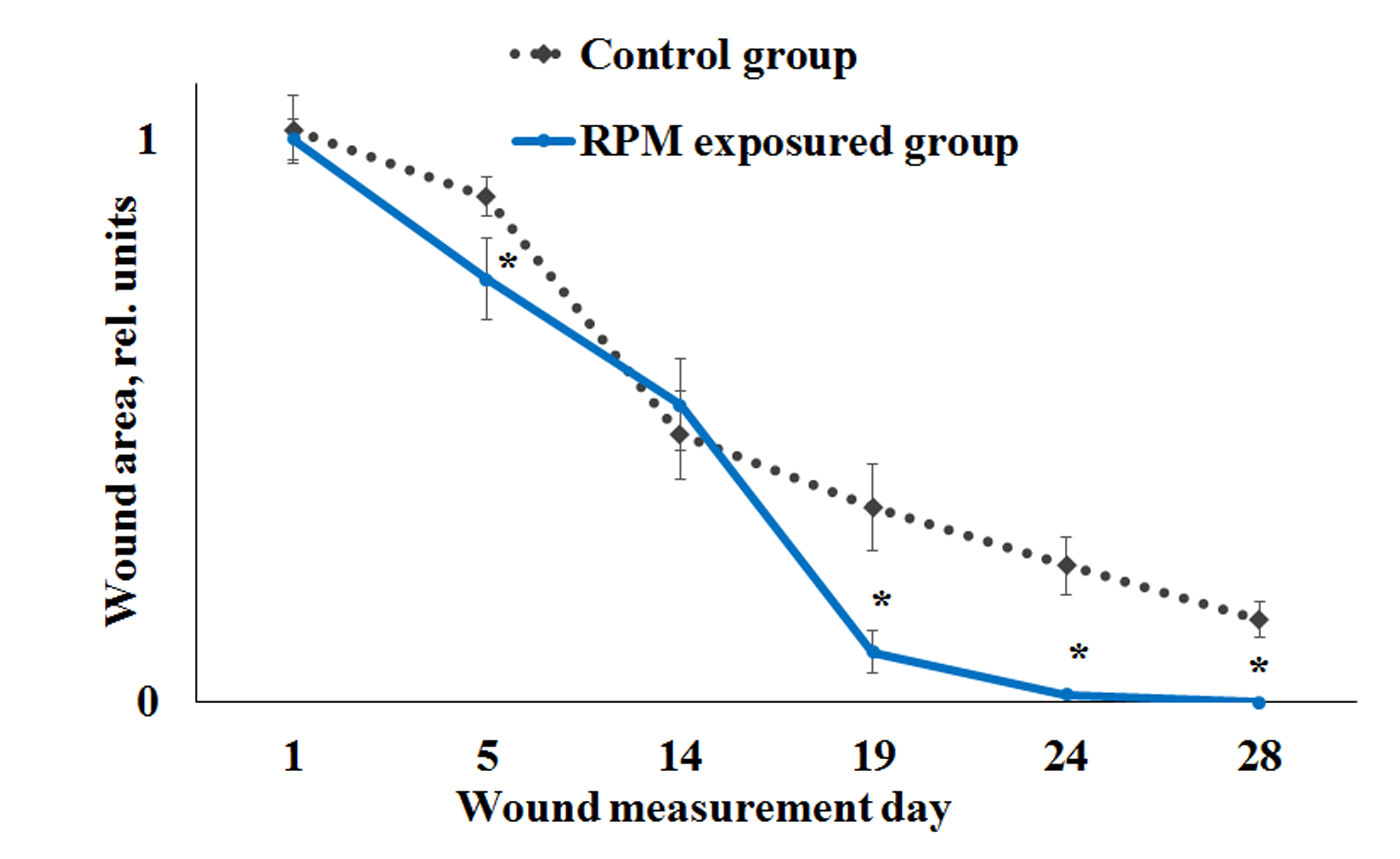
Figure 6 - Dynamics of reduction of burn wound surface in rats irradiated with nanosecond RPMs with pulse repetition rate of 8 Hz and intensity of 140 W/cm2
Note: * – differences are statistically significant in relation to the indicators of the control group of animals
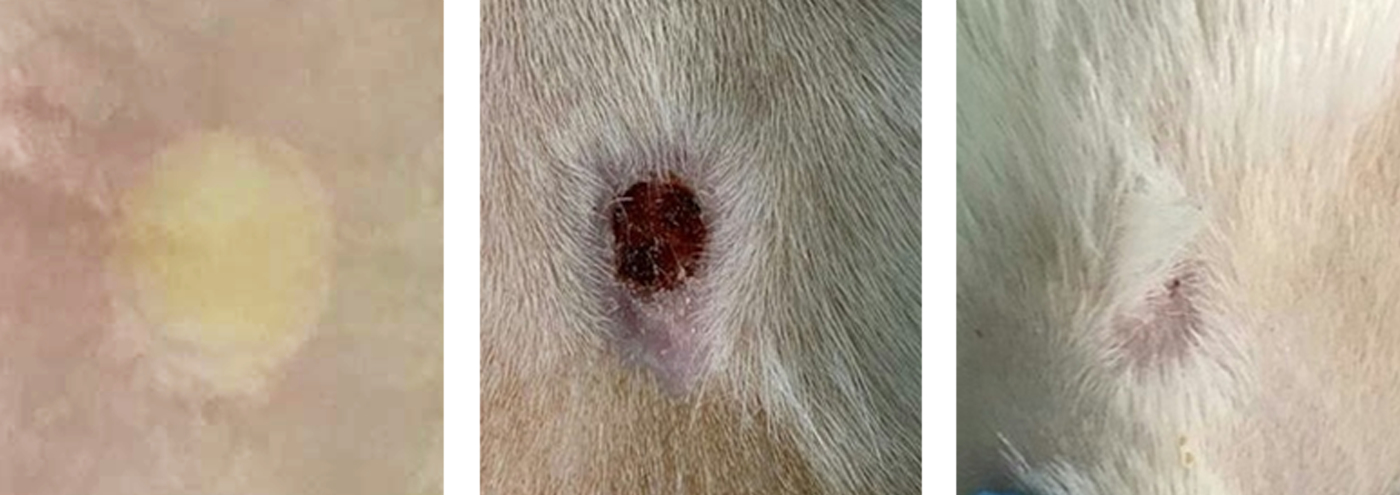
Figure 7 - Photos of the healing process of skin burn wound in the control and irradiated rats for the 1st day, the 19th day, and 30th day of the experiment
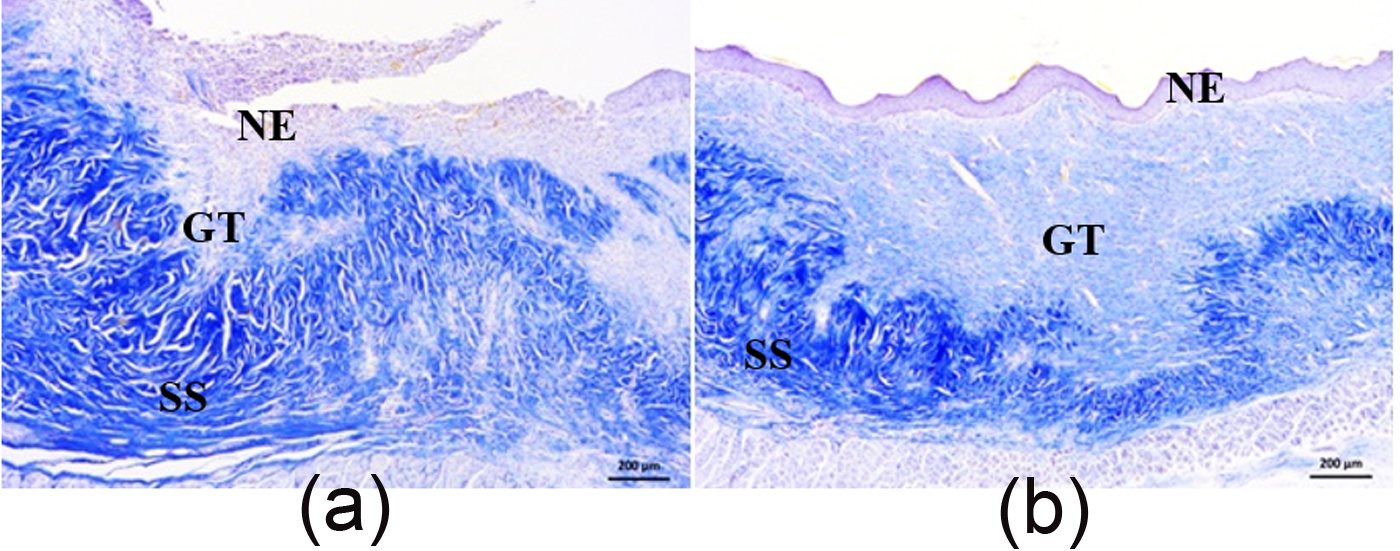
Figure 8 - Cross sections of the central region of burn wounds of the skin of rats of the control (left) and experimental (on right) groups on the 30th day of healing. Staining with the modified azan method
Note: NE - newly formed epidermis, GT - granulation tissue, SS - reticular layer of the dermis
Table 1 - Indicators of hematological analysis of red blood of Wistar laboratory rats on days 1, 5, 12 and 19 after modeling thermal injury
| 1 | 2 | 1 | 2 | 1 | 2 | 1 | 2 | 1 | 2 |
- | WBC (×109/l) | WBC (×109/l) | Gran (×109/l) | Gran (×109/l) | RBC (×1012/l) | RBC (×1012/l) | Hct, % | Hct, % | Hgb, g/l | Hgb, g/l |
1 day | 9.8±2.1 | 10.6±1.3 | 2.9±0.5 | 4.1±0.8 | 8.3±0.2 | 8.3±0.5 | 53.2±0.9 | 48.6±2.5 | 131±2.4 | 121.2±6.7 |
5 day | 7.7±1.0 | 9.3±1.7 | 2.5±0.4 | 4.2±0.1 | 7.8±0.5 | 8.6±0.2 | 52.6±1.5 | 52.3±0.5 | 130±3.7 | 130.8±1.7 |
12 day | 7.7±0.7 | 10.2±0.5 | 2.9±0.5 | 4.1±0.2 | 7.8±0.2 | 8.6±0.2 | 50.6±1.2 | 53.0±0.9 | 145.6±2.4 | 131.5±2.7 |
19 day | 9.8±1.1 | 10.7±1.1 | 2.7±0.4 | 3.9±0.7 | 9.3±0.3 | 8.8±0.3 | 53.06±3.9 | 54.2±1.1 | 142±10.4 | 132.5±3.1 |
Note: the results obtained are presented as the arithmetic mean and standard error of the arithmetic mean (M±m); 1 (n=10) – indicators of the control group, 2 (n=10) – indicators of rats irradiated for 4 days with nanosecond RPMs with pPFD (peak power flux density) of 140 W/cm2 and pulse repetition rate of 8 Hz
Such an increase may characterize the processes of stimulation of tissue regeneration at the site of injury, providing protection against the development of infection, as well as participation in maintaining blood flow and trophism of damaged tissues. In experimental animals, already in the first hours after the formation of a burn, microwave pulses have a stimulating effect on the regenerative processes in the wound and cause a decrease in the inflammatory response.
What is the nature of the effect of nanosecond microwave pulses on skin wounds? To answer this question, a computer simulation of the parameters of the microwave effect was carried out.
Computer simulation (Fig. 9 and 10) was carried out using the CST Studio Suite software package. The results showed that the directivity of the antenna (Fig. 9(a)) in open space is 15.3 dBi. The power flux density (PFD) at a distance of 20 cm from the edge of the horn in the center of the main lobe is approximately 200 W/cm2.
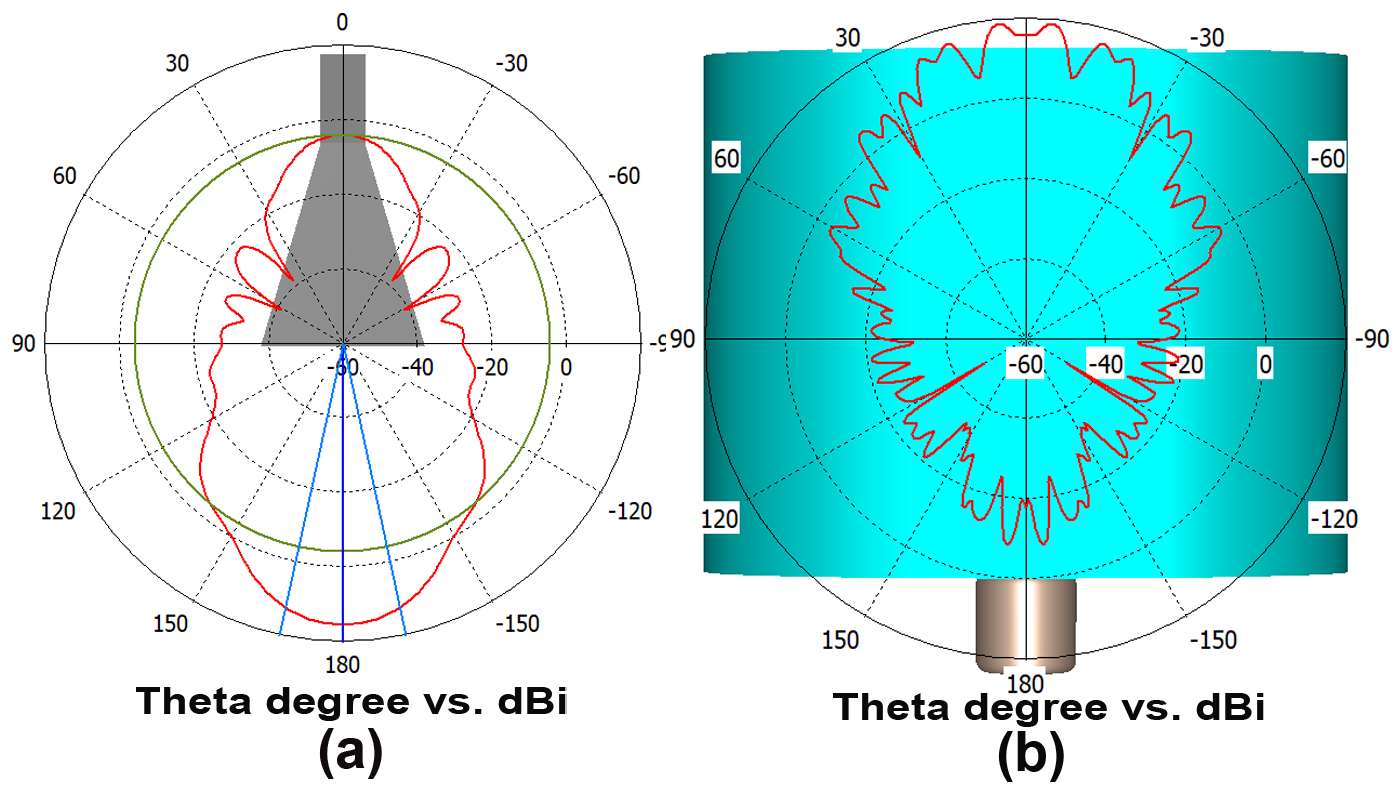
Figure 9 - Radiation patterns of a pyramidal antenna in polar coordinates
Note: (a) - radiation into open space, (b) - radiation in the chamber
In computer simulation, an object similar to the body of a rat was used, the shape of which is close to elliptical, consisting of two layers with specified characteristics of skin and muscle tissue. As was determined later, this simplification did not affect the calculation results, but significantly accelerated the calculation process itself.
The data on the properties of the skin and muscle tissue of rats used in the calculations were taken from , , .
Research related to implantable antennas for medical telemetry investigated the electrical properties of rat skin samples.
For dielectric measurements in the frequency range from 200 MHz to 20 GHz, an Agilent 85070E dielectric probe set and a PNA E8362B series network analyzer , were used. For each of the samples, a detailed construction of the frequency dependence was carried out with a total of 1800 points per graph. The results of the article were compared with studies , which have some difference towards somewhat lower relative permittivity and conductivity. It also explains that this difference is within the margin of error of the measurement and may be due either to the difference in age (100-day-old rats vs. 70-day-old rats) or to a slight loss of water during the measurement. Tissue water content decreases with age, causing a decrease in both relative permittivity and conductivity.
The process of propagation and absorption of microwaves in the experiment can be understood from the picture of the instantaneous distribution of the electric field strength in the longitudinal plane (Fig. 10(a)). The average value of the power flux-density is shown in Fig. 10(b).
Let us consider in more detail the area near the boundary of a biological object. Fig. 11 shows the rate of decrease in power flux density along the axis of wave propagation.
The test sample is located in the chamber behind the screen, and a transverse electromagnetic wave is incident on its surface (Fig. 10(a)).
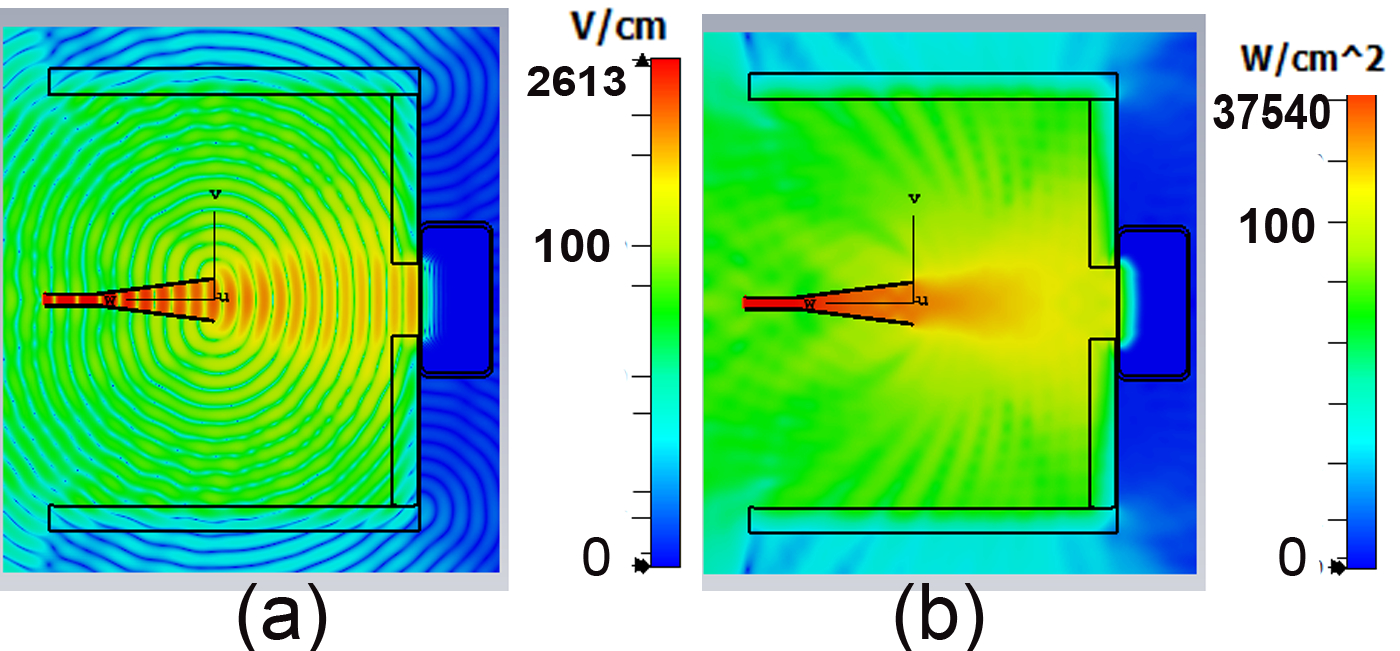
Figure 10 - (a) a picture of the formation of an electromagnetic wave in the chamber, (b) is a graph of the power flux density distribution in the horizontal plane
The coefficient of reflection from the body boundary can be written:
Writing in terms of the permittivity, we obtain:
To simplify the reasoning, the mismatch on the opposite side of the body is not taken into account. The greatest absorption of microwave power occurs in a thin layer of rat skin within 2 mm, and then it decreases in the muscle tissue.
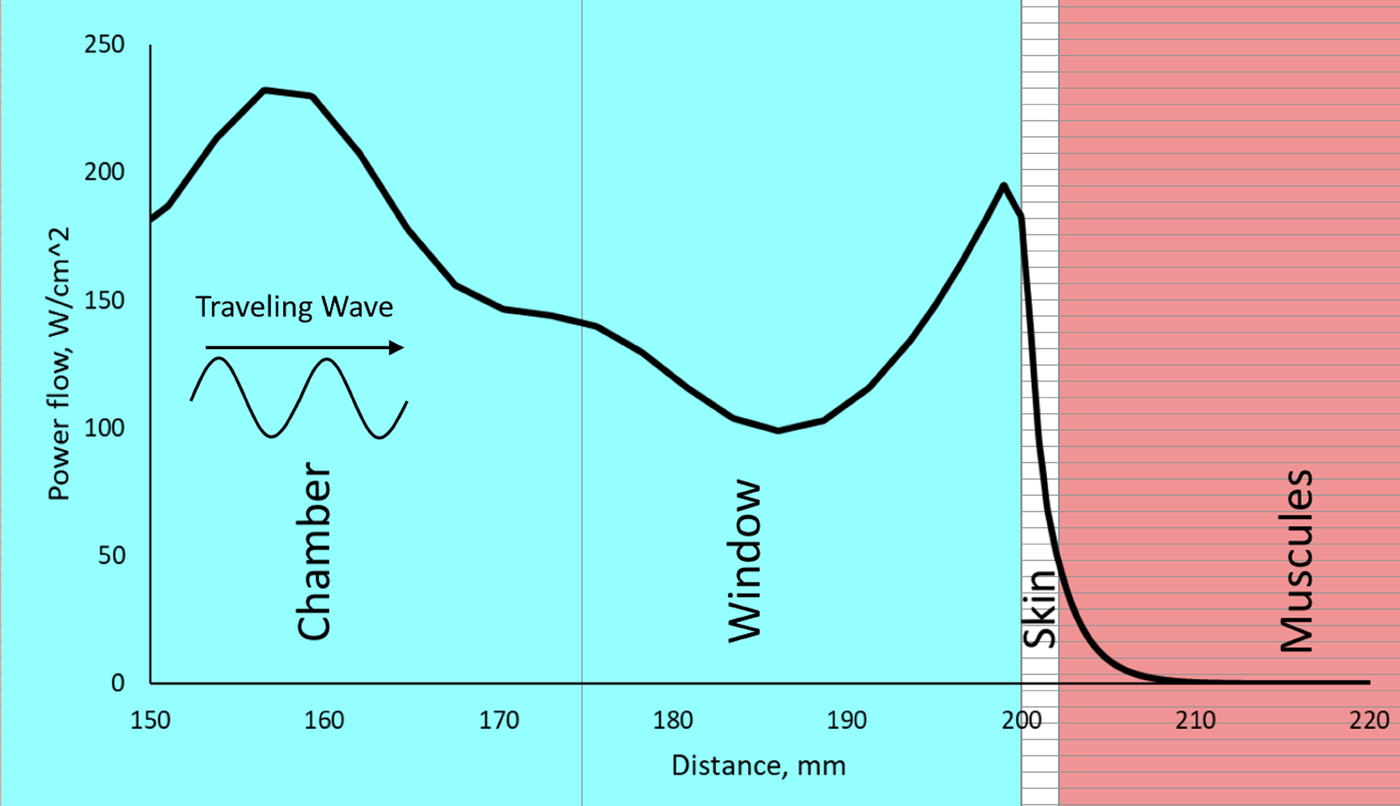
Figure 11 - The power flux density distribution on the system axis along the direction of the incident wave. On the horizontal axis, 0 corresponds to the edge of the horn antenna
To estimate the absorption of an electromagnetic wave in a layer of biological tissue L, one can use the simplified formulas .
Where α is the absorption constant, ε' and ε'' are the real and imaginary parts of the permittivity, σ is the conductivity of the medium, ε0 is the electrical constant, λ0 and f are the wavelength in free space and the frequency, respectively, l is the depth. Substituting into these expressions the data from , , for rat skin ε' = 22 and ε'' = 9.55 and for muscle tissue ε' = 49 and ε'' = 20 we obtain attenuation values close to computer modeling (Fig. 11).
4. Results and discussion
Thus, biological testing of a source of nanosecond microwave pulses demonstrated a significant stimulation of the healing of thermal burns of the skin of rats. This was confirmed by a rapid decrease in the area of the wound surface, as well as by histological analysis of the skin of experimental animals. At the same time, a number of circumstances can be noted that explain the results of the study.
The work showed that the effect of RPMs on the body did not enhance the inflammatory reactions that can occur during thermal injury, and this was confirmed by hematological parameters that were within the physiological norm. An example of this was shown in the works of Bessonov
and Lushnikov . They showed that low-intensity electromagnetic radiation of extremely high frequency has an anti-inflammatory effect, and also modifies the immune status of the body, increases its adaptive capabilities, improves microcirculation in damaged tissues, thereby activating and accelerating reparative regeneration.In addition, our previous works showed that the wound healing effect depended on the exposure mode, and the greatest effect was observed at a pulse repetition frequency of 8 Hz
, , . These results are consistent with the work of Adey (1980) , where the existence of certain “frequency energy windows” was described. In accordance with this work, the greatest biological effect is exerted by radiofrequency radiation with pulse repetition frequencies in the range of 6-16 Hz and a very specific, optimal intensity. At higher or lower intensities, the effect is significantly reduced or disappears completely. Apparently, a peak PFD of 140 W/cm2, which was used in this work in combination with a pulse repetition frequency of 8 Hz, turned out to be the optimal exposure mode. In accordance with Adey's model (Adey, 1980) , physiological processes mediated by Ca2+ ions are subject to the biological effects of electromagnetic radiation. This may be confirmed by other results demonstrating that exposure to electromagnetic radiation can enhance calcium-calmodulin binding in cells. This activates NO synthetase and promotes increased production of nitric oxide, which ensures expansion of capillaries and, accordingly, increased blood microcirculation . It was previously shown that the effect of RPM's on mouse liver mitochondria changes the content of reactive oxygen species (ROS) . Therefore, we cannot exclude the possibility that irradiation of wounds with RPM's, in addition to ROS, can similarly produce NO, which accelerates the process of epidermal regeneration and contributes to a more pronounced manifestation of scab rejection .Typically, in vertebrates and humans, damaged tissue is replaced by fibrous connective scars, which are relatively inelastic and unaesthetic. An important result of stimulation of burn regeneration under the influence of RPM's in this work is the absence of a scar. It is known from the literature that to fully restore the morphological characteristics of the skin, a complex interaction between different types of cells through cytokines and the neurovascular system is necessary . That is, a niche with the correct secretome must be formed to ensure the possibility of cell proliferation in the wound area [44]. Perhaps RPM's is capable of acting on the regenerative niche, stem cells and their signaling molecules in the area of damage, which is indirectly confirmed by our data on the effect of RPM's on the proliferation of various cells, including stem cells , , , .
5. Conclusion
The results clearly showed that the device described in the work is promising for further research into its ability to stimulate regeneration. Apparently, a similar stimulating effect can be realized for wounds of various etiologies, with the possibility of extrapolating the data to humans. It is likely that thermal wounds of various nature in people not exceeding 10% of the total skin can be restored in a short time with a positive result. Moreover, rejection of the scab at the site of the burn wound implies restoration of the skin without post-traumatic scars. Therefore, as a result of an easy series of non-invasive RPM's procedures, patients will not be at risk of adverse consequences, since low-intensity RPM's do not exceed hygienic safety levels for exposure to the body (SanPiN 2.2.4/2.1.8.055-96., 1996). Moreover, given that electromagnetic pulses can reduce pain at the wound site , the method can find wide application in medicine. Successful use of this approach will require additional research to clarify RPM's exposure parameters (peak PFD, pulse repetition rate, number of pulses applied and repeated exposure sessions), as well as development of the technical requirements necessary for the production of physical therapy equipment.